The Genetic Code
Asimov's "The Genetic Code" excerpts detail the historical understanding of inheritance, the discovery of DNA and RNA, their structure, the Watson-Crick model of replication, and the concept of mutations.
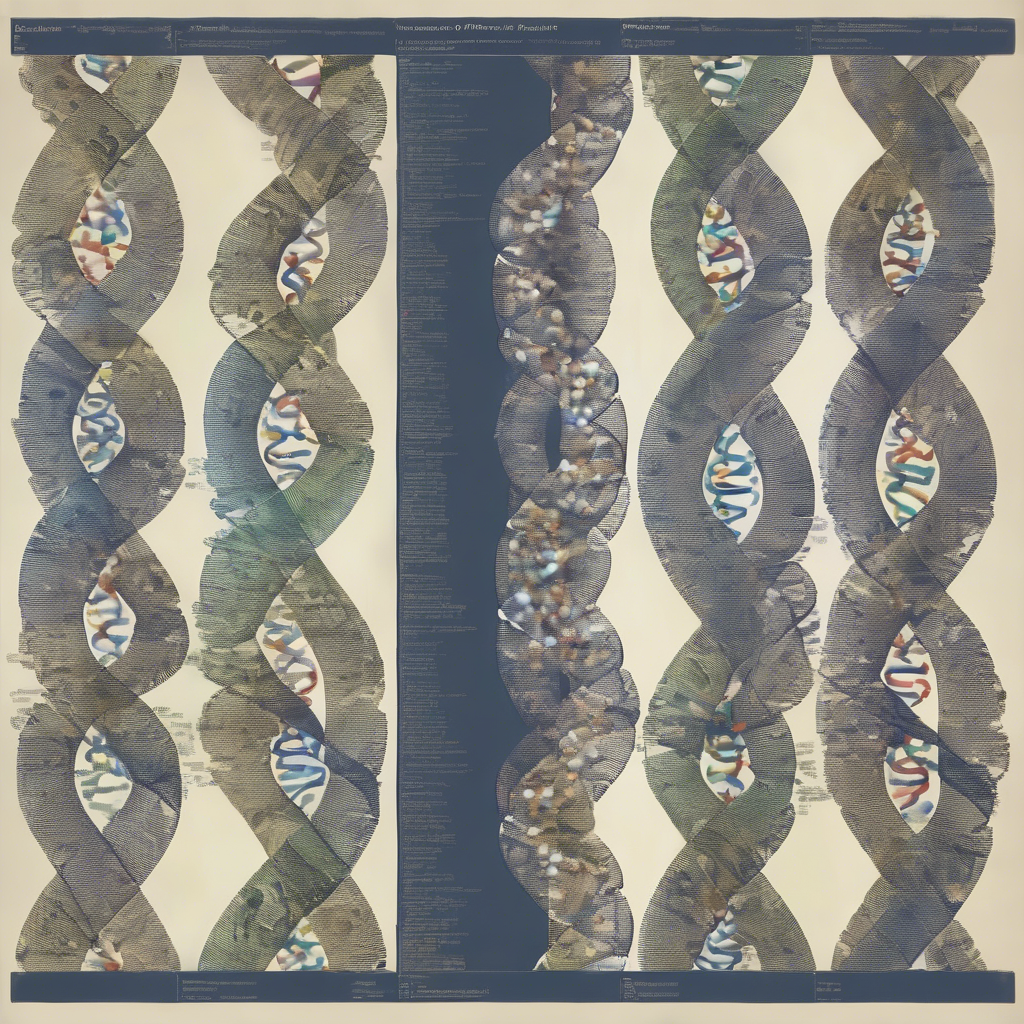
Imagine a time, not so long ago in the grand scheme of things, when the very secret of life, the way one generation passed its traits to the next, was a mystery. For centuries, people observed that children resembled their parents. It was plain to see, in the curve of a nose or the colour of hair, that something was being handed down. Even the less visible qualities, like a talent for music or a certain quickness of mind, seemed to follow family lines. This led to deep-seated beliefs about good families and bad families, notions that shaped societies in profound ways.
But observation alone could only take us so far. It was like watching the final score of a game without knowing the rules. To truly understand how these characteristics were inherited, a new approach was needed, one that moved beyond simple seeing to careful watching and thinking. It was in the 1860s that a quiet monk, Gregor Mendel, began to perform simple yet profound experiments with pea plants. By carefully crossing different varieties and recording the traits of their offspring, he laid the groundwork for a new science, the science of genetics. He showed that inheritance followed certain rules, that both parents contributed equally, and that there were factors, later called genes, which determined these traits.
The understanding took another leap forward with the study of cells, the tiny units that make up all living things. Scientists peered through microscopes and discovered a central part of the cell called the nucleus. Within this nucleus, they saw thread-like structures that took up colour when stained, and so they were named chromosomes, from the Greek word for “colour” and “thread”. These chromosomes seemed to play a crucial role when cells divided, ensuring that each new cell received a complete set. It was a natural thought to wonder if these carefully distributed chromosomes held the key to the inherited characteristics not just of single cells, but of whole organisms. After all, every complex being started as just one cell. The equal contribution of mother and father through the egg and the much smaller sperm, which contained little else besides these chromosomes, further suggested their importance.
But knowing that chromosomes were involved was not the end of the story. Scientists wanted to know what these chromosomes were made of. The chemical study of living tissues revealed that while water was the main component, the rest was made up of complex compounds unlike those found in the non-living world. Among these were proteins, which were known to be large and varied. However, experiments beginning in the 1940s pointed to another class of molecules, the nucleic acids, as the true carriers of the genetic code. The protein seemed to be more like the automobile, while the nucleic acid was the driver.
This realization sparked intense interest in the chemical structure of nucleic acids. It turned out they contained phosphorus, which was unusual for biological molecules. This phosphorus was part of phosphate groups, which gave nucleic acids their acidic properties. Scientists also discovered sugar groups within them, specifically ribose and deoxyribose. These two sugars became the defining difference between the two main types of nucleic acid: ribonucleic acid (RNA) and deoxyribonucleic acid (DNA). DNA was found mainly in the nucleus, within the chromosomes, while RNA had a wider distribution.
Further investigation revealed nitrogen-containing rings most called purines and pyrimidines as other crucial components. Two purines, adenine and guanine, and three pyrimidines, cytosine, thymine, and uracil, were identified. Interestingly, adenine, guanine, and cytosine were found in both DNA and RNA, while thymine was specific to DNA and uracil to RNA. It was like finding different sets of letters in two related alphabets.
The puzzle started to come together when scientists understood how these parts linked up. Levene proposed that a sugar, a phosphate group, and a purine or pyrimidine base combined to form a nucleotide. These nucleotides then joined together in long chains, forming polynucleotides, with a sugar-phosphate backbone and the bases sticking out. DNA had deoxyribose sugars, while RNA had ribose sugars. This chain-like structure was somewhat similar to the polypeptide chains of proteins, but with only four different bases instead of the twenty-odd amino acids. This raised a crucial question: how could just four “words” in the nucleic acid code carry enough information to build the complex machinery of life, which was built from proteins with many more “words”?
Early assumptions that nucleic acids were small and repetitive, like a simple tetranucleotide, were challenged by new findings showing they could be very long and varied. The work of Chargaff revealed that the bases were not present in equal quantities and that their ratios differed between different organisms. This suggested a far more complex and informative molecule than a simple repeating unit.
The true breakthrough came in the early 1950s with the proposal of the double helix structure of DNA by Watson and Crick, building on X-ray diffraction work by Wilkins. This model showed two polynucleotide chains winding around each other, held together by hydrogen bonds between the bases in the center. The crucial insight was that adenine always paired with thymine (or uracil in RNA), and guanine always paired with cytosine. This explained Chargaff’s rules about the equal amounts of these bases.
This double helix structure immediately suggested a mechanism for how genetic information could be copied during cell division. If the two strands separated, each could serve as a template to build a new complementary strand. This “semi-conservative” replication, where each new DNA molecule consisted of one old and one new strand, was later supported by elegant experiments.
The discovery of enzymes like those by Ochoa and Kornberg showed how these polynucleotide chains could be built in the lab. It also revealed the different ways RNA and DNA might be formed, with DNA replication appearing to require a template, fitting its role as the stable carrier of genetic information. While RNA could also replicate in some viruses, DNA seemed to be the primary blueprint in cellular life.
But if DNA held the ultimate code, what was the role of RNA? The fact that the amount of RNA varied between cells and their activities hinted at a more dynamic role than simply being a copy of DNA. The stage was set for understanding how the information stored in the seemingly simple sequence of four bases in DNA could direct the creation of the vast array of proteins that carried out the work of the cell. The story was building towards the realization that RNA served as a messenger, carrying the instructions from the DNA in the nucleus to the protein-making machinery in the cytoplasm. The simple language of the genetic code, written in just four letters, was about to be translated into the complex and diverse world of proteins. The journey from observing family resemblances to understanding the intricate dance of DNA and RNA was a testament to human curiosity and the power of scientific investigation.